Flapping Wings And The Science Of How Bees Can Fly
Jerry Seinfeld launched his career with Bee Movie, an insect-themed animated feature that took the world by storm in 2007. It posed the quandary – that supposedly, according to all known laws of aviation, bees should not be able to fly. Despite this, the bee flies anyway, because bees don’t care what humans think is impossible.
The quote isn’t easily attributed to anyone in particular, but is a cautionary tale about making the wrong assumptions in an engineering context. Yes, if you model a bee using the same maths as an airliner, of course you’ll find that it shouldn’t be able to fly. Its tiny wings can’t possibly generate enough lift to get its body off the ground. But that’s because the assumption is an erroneous one – because bees don’t fly in the same way planes do. Bees flap their wings. But that’s just the beginning. The truth is altogether more complex and interesting!
Flapping Wings and Dynamic Stall
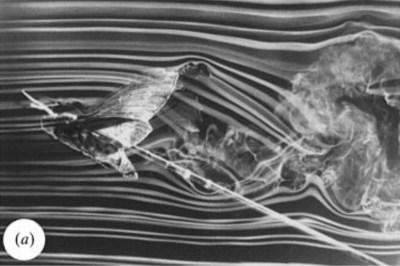
Regular planes have fixed wings that are, for all intents and purposes, relatively rigid. There is some structural flexibility, but from an aerodynamic standpoint, it doesn’t have a significant effect. These wings generate lift when moving through the air at speed, thanks to their airfoil shape. Increase the angle of the wing relative to the airflow, for example, by pitching up the aircraft, and the wing will generate more lift. This angle is called the angle of attack. Increase it too far, and the flow will separate from the wing, and it will stop producing lift entirely. This is called a stall. Without lift, planes fall out of the sky.
Bees, like birds, and many insects, don’t have fixed wings – instead, they flap their wings to generate both propulsion and lift. The wings are flapped in an incredibly complex motion, with the wing rotating throughout the downstroke and upstroke in order to maximise efficiency. The key to creating high lift with a flapping wing is down to a variety of complex fluid mechanisms.
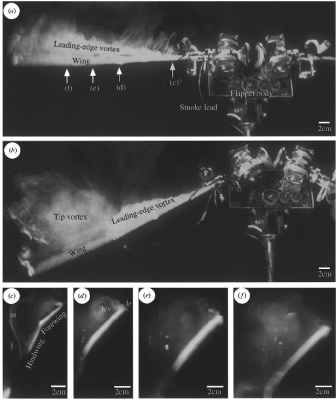
The first is the generation of a strong leading edge vortex through a phenomenon known as dynamic stall, or absence of stall. This is where the wing is at an incredibly high angle of attack on the downstroke and upstroke, which causes the airflow over the wing to seperate, generating a large vortex attached to the leading edge of the wing. This vortex remains attached to the wing, thanks to flow features generated along the span of the wing, in much the same way as delta wings work on aircraft. By keeping this vortex attached, the wing is able to generate high lift thanks to the pressure difference across the wing that would otherwise be absent if the vortex were allowed to dissipate.
The second is down to rotational effects. It’s possible to rotate the wing either before changing stroke direction, during change of stroke direction, or after changing stroke direction. When the wing rotates, this motion adds to the circulation in the existing vortexes around the wing. Doing this in advance of a stroke change, the added circulation in the air creates a boost to the lift generated by the wing; doing it after creates a negative lift force. Doing it symmetrically creates both positive and negative lift peaks throughout the full wingbeat. By varying the point of rotation, it’s possible to vary the lift generation on each flap of the wings.
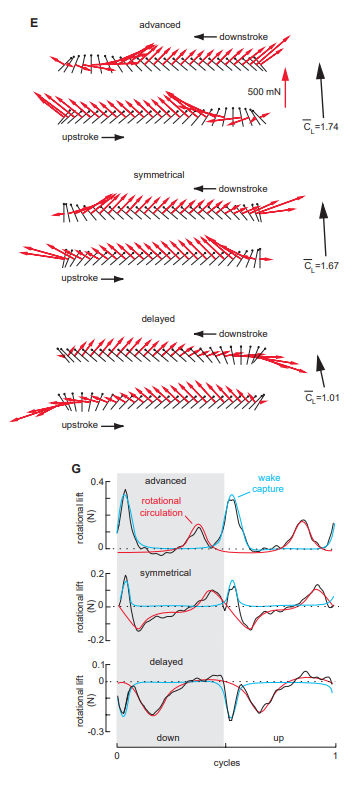
Other complex mechanisms have also been observed in various types of insects and birds, with many species displaying unique and varied flapping techniques. One technique observed in butterflies is that of wing-wake interaction. A wake is a flow regime seen in a fluid behind a moving object; most commonly observed by humans as the changing flow behind a boat travelling through water. This exists for wings in air as well. In wing-wake interaction, the motion of the wing during flapping creates an interaction between the wing’s flow and the wake shed by the previous flapping motion. As the wake in the air consists of fluid moving because of the flapping wings, interacting with this wake to generate more lift allows the insect to recapture some of the energy already expended to improve its efficiency.
Another commonly cited mechanism is the “clap and fling”, where the wings on either side of an insect are clapped together at the top of the upstroke, squeezing out air between them that helps generate thrust, before flinging apart to begin the downstroke. As the wings peel apart, they create a low-pressure zone between them that sucks in air and helps build circulation during the downstroke. However, this method is not used by all species, and only used in certain flight regimes, so is not a critical component of regular flapping wing flight.
Overall, the fluid mechanics behind flapping wing flight is incredibly complex. A basic understanding of fluid mechanics is required even to parse this very simple explainer, let alone truly dive into the topic. Flapping wing flight is still not completely understood, and is an area of ongoing research around the world. One of the reasons for this is the high level of difficulty involved in studying these phenomena.
Particularly with regard to insect flight, the flow regimes are tiny and difficult to visualise. This has led to techniques such as building robotic analogues of insect wing systems at larger scales and moving the wing surfaces through tanks of mineral oil to better see and understand the mechanisms at play. This allows techniques such as dye visualization to be used, giving insights into the complex three-dimensional flow regimes. Other work involves studying birds, which are larger and easier to observe, and running computer models. However, it’s always necessary to directly study the real thing to confirm any theory.
Regardless of the complexity, the old adage that “bees can’t fly” is provably false, and rooted more in making inappropriate engineering assumptions than any major physical paradox. As always, when running simulations, it pays to make sure you’re modelling the right thing at the get-go.
Post a Comment