How Fast is the Universe Expanding? The Riddle of Two Values for the Hubble Constant
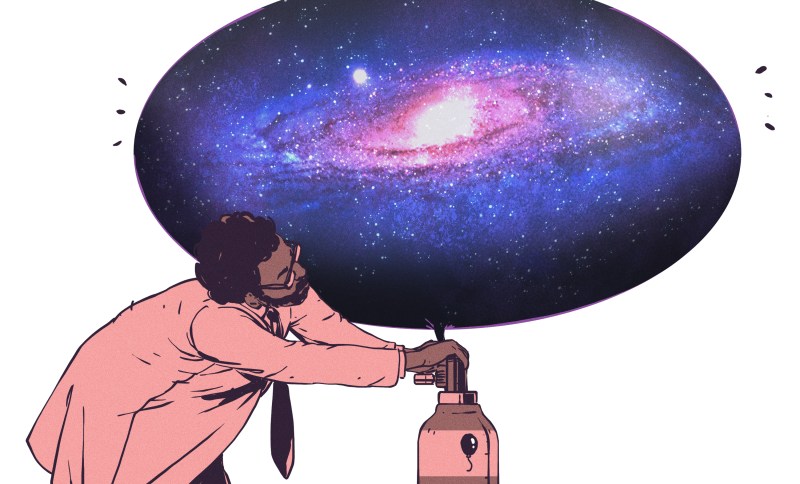
In the last decades, our understanding of the Universe has made tremendous progress. Not long ago, “precision astronomy” was thought to be an oxymoron. Nowadays, satellite experiments and powerful telescopes on earth were able to measure the properties of our Universe with astonishing precision. For example, we know the age of the Universe with an uncertainty of merely 0.3%, and even though we still do not know the origin of Dark Matter or Dark Energy we have determined their abundance with a precision of better than 1%.
There is, however, one value that astronomers have difficulty in pinning down: how fast our universe is expanding. Or, more precisely, astronomers have used multiple methods of estimating the Hubble constant, and the different methods are converging quite tightly on two different values! This clearly can’t be true, but nobody has yet figured out how to reconcile the results, and further observations have only improved the precision, deepening the conflict. It’s likely that we’ll need either new astronomy or new physics to solve this puzzle.
The Discovery of the Expanding Universe
In the 1920s Edwin Hubble used the newly built telescope at Mount Wilson Observatory to study fuzzy objects known as nebulae. Back then, astronomers were arguing whether these nebulae are clouds of stars within our Milky Way or if they are whole different galaxies. Hubble discovered stars within these nebulae whose brightness slowly fades in and out. These were known as Cepheids and previously studied by Henrietta Levitt who showed that there was a tight relationship between the star’s intrinsic brightness and the period of its variation. This means Cepheids could be used as so-called standard candles which refers to objects whose absolute brightness is known. Since there is a simple relationship between how the brightness of an object decreases with distance, Hubble was able to calculate the distance of the Cepheids by comparing their apparent and intrinsic brightness. He showed that the Cepheid stars were not located within our galaxy and that nebulae are actually distant galaxies.
Hubble also measured the velocity at which these distant galaxies are moving away from us by observing the redshifts of spectral lines caused by the Doppler effect. He found that the further away the galaxy is located, the faster it is moving away from us described by a simple linear relationship.
The parameter H0 is what is known as the Hubble constant. Later the Belgian priest and physicist Georges Lemaître realized that the velocity-distance relationship measured by Hubble was evidence for the expansion of the Universe. Since the expansion of space itself causes other galaxies to move away from us we are not in any privileged location but the same effect would be measured from any other place in the Universe. An effect that is sometimes illustrated by drawing points on a balloon, when it is inflated the points move away from each other at a speed that depends on their distance. It is also better not to think of the cosmological redshift as being caused by a real velocity as the parameter v
in the above equation can easily exceed the speed of light.
Since astronomic distances are commonly measured in Megaparsec (Mpc), which is equal to 3.26 million light-years, the Hubble constant is expressed in (km/s)/Mpc. The value of H0 is about 70 (km/s)/Mpc which can also be expressed as 7%/Gyr, meaning that the distance between two objects will increase by 7% after a billion years.
The Hubble Constant is Not Constant
Even though we speak of the Hubble constant it is a bit of a misnomer since its value is changing over time. We call this the Hubble parameter H(t) while H0 is simply the value of H(t) today. We now know that the expansion of the Universe is accelerating, so what does this mean for the Hubble constant? One might think that it will get bigger but actually it is decreasing, which can be shown with a little bit of math. We can express the Hubble parameter using the distance between two points and its time derivative
:
If we have an accelerated expansion , we get
and thus
. This means H(t) is decreasing with time. The velocity of any galaxy will increase over time because it is further away. If we look at a fixed distance, however, the velocities of different galaxies that will pass by this point will decrease over time.
How do we actually know that we live in an accelerating Universe? The proof for this came from the measurement of the redshift of distant supernovae made in the late 1990s. Similar to Cepheids, supernovae of Type 1a can be used as standard candles (i.e. their distance can be derived from their apparent brightness). Since exploding stars are generally very bright objects they can be seen from very far away.
Looking at very distant supernovae also means looking far into the past, so when the Hubble constant is changing it will have had a different value when the light from that supernovae started traveling towards us. When plotting the distance vs the redshift of supernovae one will thus see a deviation from the linear relation of the Hubble–Lemaître law for high redshifts. In the 1990s, astronomers expected to see evidence for a decelerating Universe as they thought the expansion should be slowed down due to the gravitational pull exerted by matter. Surprisingly, they found an accelerated expansion which was evidence for another form of matter or energy that acts repulsively.
Einstein originally introduced such a force to his equations of General Relativity known as the cosmological constant, denoted by the Greek letter Λ (Lambda). Ironically, it was introduced to generate a static Universe so Einstein abandoned the idea (“my biggest blunder“) when Hubble discovered the expansion of the Universe. Later the term Dark Energy was coined for the force that drives the accelerated expansion.
The Echo of the Big Bang
How do we tell how far away other stars are anyway? Astronomers have constructed a cosmic distance ladder that successively increases distance measurements using different methods. At the base of the ladder are nearby stars whose distance can be directly determined through measurements of the parallax — the apparent shift of an object’s position due to a change in an observer’s point of view. This measurement can then be used to calibrate the distance of Cepheids which then are used to calibrate the distance to Type 1a supernovae, which have brightnesses that depend on other physical properties.
Besides the distance ladder measurements described above, there are also other ways to determine the Hubble constant. One of the most precise measurements of the properties of our Universe comes from the observation of the cosmic microwave background radiation (CMB). The CMB was accidentally discovered by the radio-astronomers Penzias and Wilson after they ruled out that the signal they saw was caused by pigeons nesting in their antenna. This omnipresent source of electromagnetic radiation that peaks in the microwave region was created about 380,000 years after the Big Bang. Before that, the Universe was an opaque plasma as light did constantly bounce off free electrons and protons. Once the plasma had cooled down to about 3000K, electrons combined with protons to form neutral hydrogen atoms and light could travel freely thus the Universe became transparent.
This light which has been redshifted due to the expansion of the Universe can now be observed as the CMB. Since CMB photons are moving freely after they were last scattered they contain a snapshot of the Universe as it looked 380,000 years after the Big Bang. Through measurements of the CMB and the comparison with cosmological models, it is thus possible to extract important parameters like the aforementioned amount of Dark Matter and Dark Energy, or the Hubble constant.
Is the Universe Expanding Faster Than It Should?
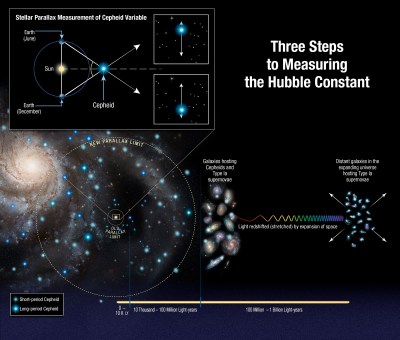
Credit: NASA, ESA, A. Feild (STScI), and A. Riess (STScI/JHU), CC BY 4.0
Currently, the most precise measurement of the CMB was performed by the Planck satellite. Its observation agrees with the current cosmological standard model, the ΛCDM model, where Λ stands for Dark Energy in form of the cosmological constant and CDM for Cold Dark Matter. The Hubble constant derived from the Planck measurement is H0 = (67.4 ± 0.5) km/s/Mpc.
However, distance ladder measurements give a value that is about 10% higher. The most precise value, in this case, was derived by the SH0ES team which used the known distance of nearby Cepheids in the Milky Way and Large Magellanic Cloud to calibrate the distance of extragalactic Type 1a supernovae as illustrated in the picture. Compared to the Planck measurement they arrive at a significantly higher value of H0 = (74.03 ± 1.42) km/s/Mpc. The tension between these two values is 4.4 standard deviations which corresponds to a probability of <0.001% as being due to chance.
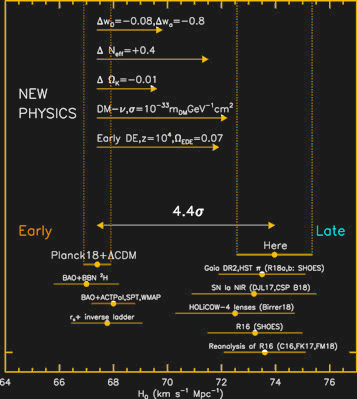
Credit: A. G. Riess, et al.
Of course, many people have tried to pin this discrepancy down to any unaccounted errors in either of the experiments but without success. Also, the discrepancy is not only between these two experiments but there are other distance ladder measurements that all point to a higher value of H0.
Making the whole situation even stranger, the CMB measurement by Planck has recently been confirmed by the Atacama Cosmology Telescope which measured a Hubble constant that is consistent with Planck’s value. In addition, CMB measurements are backed by observations of so-called baryon acoustic oscillations (BAO) combined with other astronomical data. In general, one can observe the trend that the values of H0 derived from the early Universe (CMB, BAO) are lower than those from the distance ladder measurements which use objects with much lower redshift and thus capture a more recent state of the Universe.
An important point is that the CMB measurement is model-dependent meaning that H0 is derived under the assumption that the ΛCDM model describes our Universe. So an exciting explanation for the discrepancy would be new physics beyond the current cosmological standard model. Among the many new physics interpretations for the H0 discrepancy is the idea that Dark Energy is not simply a constant but also time-dependent. Other theories include interacting Dark Matter or new relativistic particles. However, as can be seen in the figure none of these ideas can completely resolve the Hubble tension.
New Techniques to Measure Cosmic Expansion
Other techniques for determining the Hubble constant include the measurement of gravitational-lensing time delays. Strong gravitational lenses can create multiple images of an object located behind them. Since the images have different light paths there is also a time delay at which these images arrive which can be measured when the object varies in brightness. By modeling the gravitational potential of the lens and knowing the redshift of both the lens and the source, it is possible to extract the Hubble constant from this time-delay measurement. The H0LICOW (H0 Lenses in COSMOGRAIL’s Wellspring –astrophysicists have a weakness for wacky acronyms) collaboration has recently used this method to determine the Hubble constant, and their value is consistent with the distance ladder measurements and in tension with the CMB result.
In the future, completely independent measurements of the Hubble constant may shed more light on this mystery. One of them is the use of gravitational waves “standard sirens”. In this case, the absolute distance can be directly determined from the gravitational-wave measurement while the redshift is determined from simultaneous observation of electromagnetic radiation. The advantage of using gravitational waves is that the absolute distance of the source can be directly determined without any intermediate distance measurements. So any systematic error that we might have in the cosmic distance ladder will not influence the result.
The method was used to extract the Hubble constant from the gravitational-wave events GW170817 and GW190521, however, due to the large error bars, the results are consistent with both CMB and distance ladder measurements. Fortunately, the uncertainty will shrink as more and more gravitational-wave events are detected and so we will likely be able to tip the favor for either the high or low H0 value in coming years.
On the one hand, the Hubble tension is an annoying inconsistency in our otherwise well-confirmed understanding of the Universe. On the other hand, it might be an exciting glimpse of new physics. So let’s keep the hopes up that future observations will solve this puzzle and lead to new revelations.
Post a Comment